F3-Isoprostanes and F4-Neuroprostanes: Non-enzymatic Cyclic Oxygenated Metabolites of Omega-3 Polyunsaturated Fatty Acids: Biomarkers and Bioactive Lipids
Jean-Marie Galano, Camille Oger, Valérie Bultel-Poncé, Guillaume Reversat, Alexandre Guy, Joseph Vercauteren, Claire Vigor, Thierry Durand. Institut des Biomolécules Max Mousseron (IBMM), UMR 5247, CNRS, Université de Montpellier, ENSCM, Montpellier, France. Thierry Durand Institut des Biomolécules Max Mousseron (IBMM), UMR 5247, CNRS, Université de Montpellier, ENSCM, Montpellier (France). Phone: +33 4 11 75 95 58 / Email: Thierry.Durand@umontpellier.fr Abstract: The isoprostanoids are non-enzymatic oxygenated metabolites derived from polyunsaturated fatty acids (PUFA) formed in vivo by free radical mechanism. Those cyclic oxygenated metabolites named isoprostanes (IsoPs) were originally discovered from arachidonic acid (AA, C20:4 n-6) in 1990 and since then best known as biomarkers for assessing endogenous in vivo oxidative stress (OS) in humans and animals. During the last twenty-five years, a few chemist groups have successfully synthesized these cyclic oxygenated metabolites derived from omega-3 (n-3) PUFA such as F3-IsoPs from eicosapentaenoic acid (EPA, 20:5 n-3), and F4-neuroprostanes (F4-NeuroPs) from docosahexaenoic acid (DHA, 22:6 n-3), and their availability allowed a better understanding of their potential roles as bioactive compounds but also extended their use as more specific biomarkers of OS. Accordingly, we will discuss the impact of F3-IsoPs and F4-NeuroPs generated from EPA and DHA in this review.
The discovery and study of isoprostanoids have provided a major step forward in the field of free radical research. The quantification of these oxygenated lipids has opened up new areas of investigation regarding the role of free radicals in human physiology and pathology, and appears to be the most useful tool currently available to explore the role of endogenous lipid peroxidation in human diseases. However, as explained below such lipids are not only reliable biomarkers but also exert bioactive properties. So far, evidence in favor of the bioactive role of isoprostanoids from n-6 PUFA were shown in various biological systems [6, 7, 11]. 15-F2t-IsoP will be not developed in this review (for references, see recent reviews) [6, 7, 11, 14, 15].
In 2002, new oxygenated metabolites were discovered with a furan core as the main feature. Their formation follows the initial same free radical cascade pathway of IsoPs, but a competition between the five-membered ring formation (IsoPs) and attack of further diradical oxygen lead to the competitive generation of isofurans (IsoFs) from AA [17]. It is now well described that both types of metabolites are present in most lipid matrices. Similarly, other PUFAs can also generate isofuranoid derivatives, [6] including neurofurans (NeuroFs) (Figure 3) [17, 18] and dihomo-isofurans [19] (dihomo-IsoFs) from DHA and adrenic acid (AdA, 22:4 n-6) respectively.
There are two nomenclatures proposed by Taber [20] and Rokach [21] to name such metabolites. Taber nomenclature was approved by IUPAC and will be used throughout this review. To avoid confusion, the structure of 4-F4t-NeuroP is presented in Figure 4 and will be described briefly. The ring substitution pattern with A-H, J and L is based on the common PG nomenclature (F in this case with two hydroxyls). The number as a subscript gave the number of double bonds on the two side chains (4 in this case). The t or c as a subscript determines the relative orientation of the α chain with respect to the adjacent α OH in the cyclopentane ring (t in this case). The family name NeuroP, IsoP, and PhytoP is based on the PUFAs (NeuroP from DHA in this case). Refined extraction methods, robust analysis and elucidation of chemical structures have improved the sensitivity of detection in biological tissues and fluids. The first reliable instrumentation for measurement was gas chromatography-mass spectrometry (GC-MS), and nowadays the use of liquid chromatography tandem mass spectrometry (LC-MS/MS) is gaining much attention [see recent prominent reviews, [8-10, 22, 23].
- Introduction
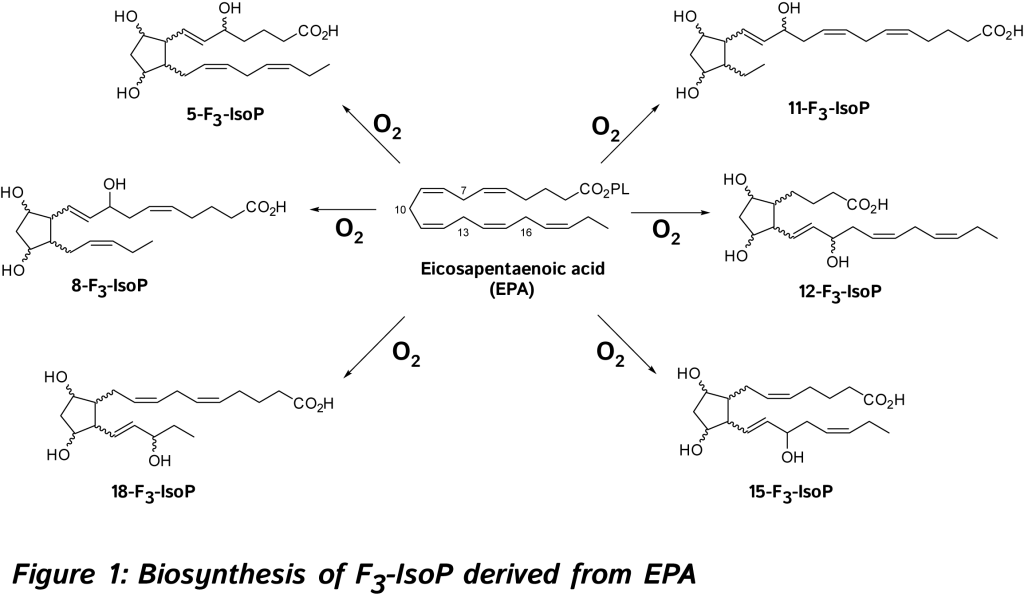
- Formation, nomenclature and quantitation of F3-IsoPs, F4-NeuroPs derived from EPA and DHA, and isofurans.
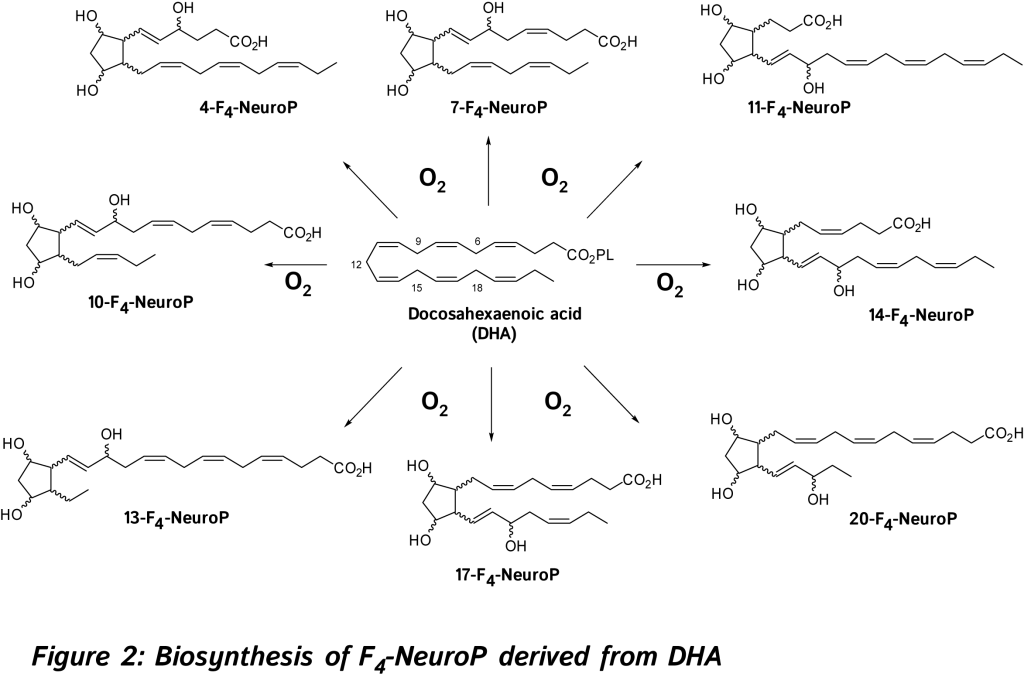
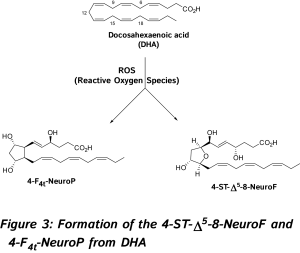
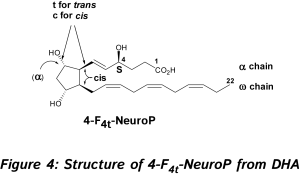
- Chemical syntheses.
- Biomarkers
- Biological activities
- Conclusion
- Ruxton, C., Health benefits of omega-3 fatty acids. Nurs. Stand., 2004 18(48): p. 38-42. (link)
- Gardner, H.W., Oxygen radical chemistry of polyunsaturated fatty acids. Free Radic. Biol. Med., 1989. 7(1): p. 65-86. (link)
- Morrow, J.D., et al., A series of prostaglandin F2-like compounds are produced in vivo in humans by a non-cyclooxygenase, free radical-catalyzed mechanism. Proc. Natl. Acad. Sci. U.S.A., 1990 87(23): p. 9383-9387. (link)
- Nourooz-Zadeh, J., B. Halliwell, and E.E. Änggård, Evidence for the formation of F3-isoprostanes during peroxidation of eicosapentaenoic acid. Biochem. Biophys. Res. Commun., 1997. 236(2): p. 467-472. (link)
- Nourooz-Zadeh, J., et al., F4-isoprostanes: a novel class of prostanoids formed during peroxidation of docosahexaenoic acid (DHA). Biochem. Biophys. Res. Commun., 1998. 242(2): p. 338-344. (link)
- Jahn, U., J.M. Galano, and T. Durand, Beyond prostaglandins - Chemistry and biology of cyclic oxygenated metabolites formed by free-radical pathways from polyunsaturated fatty acids. Angew. Chem. Int. Ed. Engl., 2008. 47(32): p. 5894-5955. (link)
- Milne, G.L., et al., Isoprostane generation and function. Chem. Rev., 2011. 111(10): p. 5973-5996. (link)
- Vigor, C., et al., Non-enzymatic lipid oxidation products in biological systems: assessment of the metabolites from polyunsaturated fatty acids. J. Chromatogr. B Analyt. Technol. Biomed. Life Sci., 2014. 964: p. 65-78. (link)
- Leung, K.S., et al., Current development in non-enzymatic lipid peroxidation products, isoprostanoids and isofuranoids, in novel biological samples. Free Radic. Res., 2015. 49(7): p. 816-826. (link)
- Galano, J.M., et al., Non-enzymatic cyclic oxygenated metabolites of adrenic, docosahexaenoic, eicosapentaenoic and α-linolenic acids; bioactivities and potential use as biomarkers. Biochim. Biophys. Acta, 2015. 1851(4): p. 446-455. (link)
- Galano, J.-M., et al., Special Issue on “Analytical Methods for Oxidized Biomolecules and Antioxidants” The use of isoprostanoids as biomarkers of oxidative damage, and their role in human dietary intervention studies. Free Radic. Res., 2015. 49: p. 583-598. (link)
- Roy, J., et al., Non-enzymatic cyclic oxygenated metabolites of omega-3 polyunsaturated fatty acid: Bioactive drugs? Biochimie, 2016. 120: p. 56-61. (link)
- Kadiiska, M.B., et al., Biomarkers of oxidative stress study II: are oxidation products of lipids, proteins, and DNA markers of CCl4 poisoning? Free Radic. Biol. Med., 2005. 38(6): p. 698-710. (link)
- An overview on isoprostane research: from chemistry to clinical medicine. Chem. Phys. Lipids. 2004. 128(1–2): p. 1-194. (link)
- Cracowski, J.L., T. Durand, and G. Bessard, Isoprostanes as a biomarker of lipid peroxidation in humans: physiology, pharmacology and clinical implications. Trends Pharmacol. Sci., 2002. 23(8): p. 360-366. (link)
- Stafforini, D.M., et al., Release of free F2-isoprostanes from esterified phospholipids is catalyzed by intracellular and plasma platelet-activating factor acetylhydrolases. J. Biol. Chem., 2006. 281(8): p. 4616-4623. (link)
- Fessel, J.P., et al., Discovery of lipid peroxidation products formed in vivo with a substituted tetrahydrofuran ring (isofurans) that are favored by increased oxygen tension. Proc. Natl. Acad. Sci. U. S. A., 2002. 99(26): p. 16713-16718. (link)
- Song, W.L., et al., Neurofurans, novel indices of oxidant stress derived from docosahexaenoic acid. J. Biol. Chem., 2008. 283(1): p. 6-16. (link)
- De La Torre, A., et al., Synthesis, discovery, and quantitation of dihomo-isofurans: Biomarkers for in vivo adrenic acid peroxidation. Angew. Chem. Int. Ed. Engl., 2014. 53(24): p. 6249-6252. (link)
- Taber, D.F., J.D. Morrow, and L.J. Roberts, A nomenclature system for the isoprostanes. Prostaglandins, 1997. 53(2): p. 63-67. (link)
- Rokach, J., et al., Nomenclature of isoprostanes: a proposal. Prostaglandins, 1997. 54(6): p. 853-873. (link)
- Arneson, K.O. and L.J. Roberts, Measurement of products of docosahexaenoic acid peroxidation, neuroprostanes, and neurofurans. Methods Enzymol., 2007. 433: p. 127-143. (link)
- Lee, Y.Y., et al., Assessment of isoprostanes in human plasma: Technical considerations and the use of mass spectrometry. Lipids, 2016. 51(11): p. 1217-1229.
- Durand, T., et al., Total synthesis of 4(RS)-F4t-isoprostane methyl ester. Tetrahedron Letters, 2000. 41(20): p. 3859–3862. (link)
- Oger, C., et al., The handy use of Brown's P2-Ni catalyst for a skipped diyne deuteration: application to the synthesis of a [D4]-labeled F4t-neuroprostane. Chemistry, 2010. 16(47): p. 13976-13980. (link)
- Guy, A., et al., Oxygenated metabolites of n-3 polyunsaturated fatty acids as potential oxidative stress biomarkers: total synthesis of 8-F3t-IsoP, 10-F4t-NeuroP and [D4]-10-F4t-NeuroP. Chemistry, 2014. 20(21): p. 6374-6380. (link)
- Jacobo, S.H., et al., Total synthesis of 8,12-iso-iPF3alpha-VI, an EPA-derived isoprostane: stereoselective introduction of the fifth asymmetric center. J. Org. Chem., 2006. 71(4): p. 1370-1379. (link)
- Quan, L.G. and J.K. Cha, Preparation of isoprostanes and neuroprostanes. J. Am. Chem. Soc., 2002. 124(42): p. 12424-12425. (link)
- Taber, D.F., P.G. Reddy, and K.O. Arneson, A potential route to neuroprostanes and isoprostanes: preparation of the four enantiomerically pure diastereomers of 13-F4t-neuroP. J. Org. Chem., 2008. 73(9): p. 3467-3474. (link)
- Zanoni, G., et al., Asymmetric synthesis of 14-A4t-neuroprostane: hunting for a suitable biomarker for neurodegenerative diseases. J. Org. Chem., 2007. 72(25): p. 9698-9703. (link)
- Barden, A.E., et al., Fish oil supplementation in pregnancy lowers F2–isoprostanes in neonates at high risk of atopy. Free Radic. Res., 2004. 38: p. 233-239. (link)
- Barden, A.E., et al., Is there a role for isofurans and neuroprostanes in pre-eclampsia and normal pregnancy? Antiox. Redox Signal., 2012. 16(2): p. 165-169. (link)
- Corcoran, T.B., et al., Are isofurans and neuroprostanes increased after subarachnoid hemorrhage and traumatic brain injury? Antioxidants & Redox Signaling, 2011. 15(10): p. 2663-2667. (link)
- Hsieh, Y.P., et al., Correlation of F4-neuroprostanes levels in cerebrospinal fluid with outcome of aneurysmal subarachnoid hemorrhage in humans. Free Radic. Biol. Med., 2009. 47: p. 814-824. (link)
- Gladine, C., et al., Lipid profiling following intake of the omega 3 fatty acid DHA identifies the peroxidized metabolites F4-neuroprostanes as the best predictors of atherosclerosis prevention. PLoS ONE, 2014. 9(2): p. e89393. (link)
- Sastry, P.S., Lipids of nervous tissue: composition and metabolism. Prog. Lipid Res., 1985. 24(2): p. 69-176. (link)
- VanRollins, M., et al., F2-Dihomo-isoprostanes arise from free radical attack on adrenic acid. J. Lipid Res., 2008. 49(5): p. 995-1005. (link)
- De Felice, C., et al., The role of oxidative stress in Rett Syndrome: an overview. Ann. NY Acad. Sci., 2012. 1259: p. 121-135. (link)
- De Felice, C., et al., F2-dihomo-isoprostanes as potential early biomarkers of lipid oxidative damage in Rett syndrome. J. Lipid Res., 2011. 52(12): p. 2287-2297. (link)
- De La Torre, A., et al., Total syntheses and in vivo quantitation of novel neurofuran and dihomo-isofuran derived from docosahexaenoic acid and adrenic acid. Chemistry, 2015. 21(6): p. 2442-2446. (link)
- Galano, J.M., et al., Isoprostanes and neuroprostanes: total synthesis, biological activity and biomarkers of oxidative stress in humans. Prostaglandins Other Lipid Mediat, 2013. 107: p. 95-102. (link)
- Serhan, C.N., N. Chiang, and T.E. Van Dyke, Resolving inflammation: dual anti-inflammatory and pro-resolution lipid mediators. Nat. Rev. Immunol., 2008. 8: p. 349-361. (link)
- Serhan, C.N., et al., Maresins: novel macrophage mediators with potent antiinflammatory and proresolving actions. J. Exp. Med., 2009. 206: p. 15-23. (link)
- Praticò, D., et al., Local amplification of platelet function by 8-Epi prostaglandin F2alpha is not mediated by thromboxane receptor isoforms. J. Biol. Chem., 1996. 271(25): p. 14916-14924. (link)
- Jamil, J., et al., Role of the non-enzymatic metabolite of eicosapentaenoic acid, 5-epi-5-F3t-isoprostane in the regulation of [(3)H]D-aspartate release in isolated bovine retina. Neurochem. Res., 2014. 39(12): p. 2360-2369. (link)
- Brooks, J.D., et al., The fatty acid oxidation product 15-A3t-isoprostane is a potent inhibitor of NFkappaB transcription and macrophage transformation. J. Neurochem., 2011. 119(3): p. 604-616. (link)
- Gao, L., et al., Novel n-3 fatty acid oxidation products activate Nrf2 by destabilizing the association between Keap1 and Cullin3. J. Biol. Chem., 2007. 282(4): p. 2529-2537. (link)
- Musiek, E.S., et al., Electrophilic cyclopentenone neuroprostanes are anti-inflammatory mediators formed from the peroxidation of the omega-3 polyunsaturated fatty acid docosahexaenoic acid. J. Biol. Chem., 2008. 283(29): p. 19927-19935. (link)
- Roy, J., et al., Nonenzymatic lipid mediators, neuroprostanes, exert the antiarrhythmic properties of docosahexaenoic acid. Free Radic. Biol. Med., 2015. 86: p. 269-278. (link)
- Le Guennec, J.Y., et al., EP2013/07546. PCT, 2013. 4 December.
- Jude, S., et al., Peroxidation of docosahexaenoic acid is responsible for its effects on I TO and I SS in rat ventricular myocytes. Br. J. Pharmacol., 2003. 139(4): p. 816-822. (link)
- Le Guennec, J.Y., et al., EP2016/079307. PCT, 2016. 30 November.
- Roy, J., et al., Non-enzymatic oxidized metabolite of DHA, 4(RS)-4-F4t-neuroprostane protects the heart against reperfusion injury. Free Radic. Biol. Med., 2016. 102: p. 229-239. (link)
- Bosviel, R., et al., DHA-derived oxylipins, neuroprostanes and protectins, differentially and dose-dependently modulate the inflammatory response in human macrophages: Putative mechanisms through PPAR activation. Free Radic. Biol. Med., 2016. 103: p. 146-154. (link)